1.IntroductionCollagen is the most widespread structural protein in higher vertebrates. It comprises about 6 of body weight in mammals and is the main means of their structural support. Collagen has a molecular weight of about 300 kDa and its molecules consist of three peptide chains that form a rod shaped, triple helix. The studies of collagen in cell and molecular biology reveal that it is an essential component of extracellular matrix in organism, but also performs important physiological functions in cell migration, proliferation, differentiation, and growth. Many kinds of diseases are related to pathological changes of collagen, including tumorogenesis and metastasis of neoplastic cells. The study of collagen interaction with photosensitizers and light is of high importance. In photodynamic therapy (PDT) of skin cancer and other superficial malignant tumors, the excitation light propagates through the skin, which is rich in collagen content. Some exogenous photosensitizers accumulate in the skin increasing the patients’ photosensitivity during photodynamic therapy, this being one of the major drawbacks of PDT. The pronounced, characteristic fluorescence of collagen in the spectral range of 370–700 nm and its alteration at different excitation wavelengths could prove valuable for fluorescence diagnosis of atherosclerotic plaque and malignant tumors. The fluorescence of collagen in the UVA (335–400 nm) and visible spectral regions has been investigated in many papers 1 2 and several attempts have been made to clarify the nature and origin of it. 3 4 Destruction of type I collagen under UVA and broadband solar simulating radiation (290–400 nm) has also been demonstrated 5 and the existence of at least four photolabile fluorescent chromophores has been shown therein. These chromophores were found to absorb optical radiation in the region between 300 and 400 nm. The alterations induced to collagen, the main connective protein in human skin, under chronic exposure to solar UV have also been well documented. 5 6 7 These studies have demonstrated collagen crosslinking, destruction of tyrosine, and phenylalanine residues, conformational change with concomitant chain degradation, and suppression of fibril formation. Fluorescence photobleaching of artery-fluorescent constituents (including collagen) and skin at 337, 476, and 514 nm laser irradiation was studied. 8 9 10 11 These studies demonstrated that the fluorescence emission of artery and skin decreased, even though the total irradiation fluence was comparable to that used for in vivo fluorescence diagnosis. Furthermore, structural modifications of hematoporphyrin-enriched collagen gels, irradiated by Ar + laser, were characterized. 12 It was suggested that the chosen system could prove appropriate to model the influence of the semisolid nature of tissues, in particular of the tumor stroma collagen, on the photodynamic effect. However, up to now the photochemical processes induced by visible light in pure collagen, as well as in collagen sensitized by more photoactive exogenous dyes, have not been extensively investigated. The study of the photochemical interaction of collagen with hypericin is of major interest, since it has been successfully applied in fluorescence diagnosis of stomach cancer 13 and PDT of superficial cancers. 14 Hypericin (HYP), a polycyclic aromatic quinone, is a natural pigment synthesized by plants of the Hypericum family as well as by some insects, fungi, and protozoa. HYP has recently received increasing attention due to its high toxicity against viruses, including HIV, under the presence of light 14 15 and its antitumor photoactivity, 16 due to a combination of several mechanisms. These mechanisms include singlet oxygen production (ФΔ≈0.5), superoxide anions formation, 17 and excited state charge transfer processes. 18 19 The photophysical properties of HYP seem to be strongly dependent on the surrounding medium. In this context its fluorescence quantum yield is ФF≈0.2 in polar organic solvents, but drops quite steeply (ФF≈0.02) in water and organic apolar solvent environment, due to extensive molecular aggregation. 20 Its photoactive properties have caused HYP to be considered as a potent photosensitizer for the photodynamic therapy and fluorescence diagnosis of cancer, as well as numerous other diseases. 15 16 The primary goal of this work was to elucidate the photophysical and photochemical processes in collagen induced both by endogenous and exogenous cromophores under laser irradiation both in the UV and visible spectral regions. In this context, we investigated laser-induced photoprocesses in collagen and demonstrated that both UV (355 nm) and visible light (532 nm) can induce irreversible damage to collagen. An important aspect that arises from the current work is that skin collagen is permanently affected by sunlight. Different kinds of photosensitizers, both endogenous and exogenous, can enhance these induced photochemical processes in collagen. In the current work HYP was used as an exogenous photosensitizer. In order to clarify the photoprocesses involved, HYP photosensitization results were compared to those obtained after collagen photosensitization with chlorin e 6 (chle 6 ). To further understand the role of collagen triple-helix structure in photochemical processes taking place among the various collagen fluorophores, formed perhaps by crosslinking, these processes in collagen were compared to those induced to gelatin (heat-denatured collagen). Gelatin has the same aminoacid chain sequence as collagen but lacks the lattice-ordered triple-helix structure. 1 2.Materials and MethodsType I collagen from bovine Achilles tendon and gelatin (Fluka Biochemica 27662 and 48724, respectively) were used. Collagen was studied in dry form, as well as in aqueous environment. The collagen samples used were composed of fibrils, 1–3 mm long. Gelatin was studied in dry granular form. HYP derived from Hypericum Perforatum, according to a standard gel column chromatography procedure, 15 was used as a sensitizer in aqueous solution. Chle 6 obtained from the Institute of Molecular and Atomic Physics (Minsk, Belarus) was also used. The samples were placed between two thin quartz slides with virtually no detectable fluorescence at 260–600 nm excitation. Photobleaching and fluorescence spectra of the samples were studied by using a computerized laser system based on a Q-switched pulsed Nd:YAG laser, with emission at 1064 nm and pulse width of about 12 ns full width half maximum (FWHM) (Figure 1). The fundamental frequency was converted into the second ( λ=532 nm, pulse energy E=15 mJ ), third ( λ=355 nm, E=5 mJ ), and forth ( λ=266 nm, E=2 mJ ) harmonics by using LiJO 3 and KDP nonlinear crystals. The generated harmonics were propagated through a dispersion prism (α=60°), and each required wavelength was time selected by spatial barriers absorbing laser beams at other wavelengths. The intensity of laser radiation at 532 and 355 nm was attenuated by using neutral density glass filters, with 50 and 75 absorption at those wavelengths. The spot of the laser beam focused on the approximately 3 by 3 mm and 0.2 mm thick samples was an ellipse of about 1.5 mm by 2 mm. By more intense focusing of laser radiation on the sample, a spot with a diameter less than 0.5 mm was formed, allowing the separation of the sample fluorescence from that of the surrounding medium. The emission from the sample was collected by a focusing lens at 90° to the laser beam. It was consequently delivered to the input slit of a scanning monochromator, equipped with a stepper-motor wavelength drive (MDR-23, LOMO, St. Petersburg; UV-Vis grating=1200 grooves/mm; bandpass=3 nm FWHM ) which together with a photomultiplier tube (FEU-79; rise time=3 ns; high voltage=1500–1900 V ) was used for detection and spectral analysis of the fluorescence signal. The photomultiplier in combination with an oscilloscope (C1-75) provided a time resolution of 80 ns. A fraction of the laser radiation was partly directed by a quartz beam splitter to a photodiode for normalization of the excitation radiation. A personal computer and a two-channel analog-to-digital converter were used for controlled scanning of the output wavelength on the monochromator, as well as for automatic data collection and processing. The emission spectra recorded by the laser spectrofluorometer were normalized by division to a correction factor determined by the use of a standard ribbon-type tungsten lamp according to a generally accepted method. 21 Fluorescence spectra were obtained by recording the mean value of fluorescence signals induced by ten consequent laser excitation pulses, for each wavelength at the monochromator exit. The parameters of laser pulses were: pulse repetition rate F=5 or 10 Hz and fluence rate P=0.1 mJ/cm 2 . Kinetics of fluorescence photobleaching were obtained by averaging fluorescence signals arriving at the data acquisition system every 2 s, for sample irradiation at F=5 or 10 Hz and average intensity of 50, 100, 150, and 200 mW/cm2 (λ exc =532 nm) or 15 mW/cm2 (λ exc =355 nm). The mean-square deviations were less than 15 for each point. A Perkin-Elmer Hitachi MPF 43B spectrofluorometer was used for registration of excitation spectra of collagen and dry gelatin. 3.ResultsUsing the experimental setup described above, the fluorescence spectra of dry collagen at three excitation wavelengths: 266, 355, and 532 nm, as well as the emission spectrum of dry gelatin at 266 nm, were obtained (Figure 2). Emission lifetime was in all cases found to be less than 80 ns, the time resolution of the laser spectrofluorometer. In Figure 2 it can be seen that the emission spectra of collagen were strongly dependent on excitation wavelength. Excitation of collagen at 266 nm produced a fluorescence spectrum with maximum at 387 nm, which redshifted to 395 at 355 nm excitation. The presence of a shoulder can be seen at 405 nm in the latter case. Finally excitation at 532 nm produced a fluorescence spectrum without structure and distinctive maximum. The maximum of gelatin fluorescence was observed at 402 nm, at 355 nm excitation. Figure 2Fluorescence spectra of dry collagen (1)–(3) and gelatin (4) excited by 266 nm (1), (4), 355 nm (2), and 532 nm (3) laser radiation. ![]() The excitation spectra of collagen were taken at 387, 405, 440, and 480 nm emission wavelengths as shown in Figure 3. The corresponding maxima were at 338, 343, and 372 nm, while for emission at 480 nm there was no maximum, but monotonic increase almost up to the emission wavelength. Figure 3Excitation spectra of dry collagen. λ emiss : 387 nm (1), 405 nm (2), 440 nm (3), and 480 nm (4). ![]() A comparison between the excitation spectrum of gelatin at 440 nm emission (Figure 4, curve 3) and that of collagen at the same emission wavelength (Figure 3, curve 3) revealed that in the case of gelatin the spectrum was shifted to longer wavelengths. There appeared to be a shoulder centered at 379 nm, while there was a maximum at 400 nm. For emission at 410 nm the excitation spectrum of gelatin (Figure 4, curve 2) presented a maximum at 363 nm. Addition of bidistilled water to collagen produced proportional quenching of its fluorescence in the 385–450 nm spectral region, while addition of HYP aqueous solution to collagen produced nonuniform quenching. The quenching of collagen fluorescence was stronger in the short wavelength region and considerably weaker in the long wavelength region of the spectrum, resulting in a change of the spectral profile. The quenching, redshift of the maximum, and broadening of the spectral form of collagen fluorescence increased, with increasing HYP concentration. The effect of different concentrations of HYP on collagen can be seen in Figure 5. After addition of 0.03 mL from 10−5 M HYP solution, the maximum of collagen fluorescence shifted from 395 to 420 nm at 355 nm excitation. A 10 nm additional shifting of this maximum was observed after further addition of 0.03 mL from 10−5 M HYP solution. Each addition of 0.03 mL from HYP 10−5 M solution to the sample increased its optical density by a value of less than 10−2, in the 355–500 nm spectral. Hence the absorption of the excitation radiation or collagen fluorescence by HYP can be considered negligible. It must also be noted that HYP emits in 580–700 nm spectral range 16 and thus the contribution of HYP fluorescence, below 575 nm, is equally negligible. During water evaporation from the collagen sample, more effective quenching and shifting of its fluorescence was observed (Figure 5). Figure 5Fluorescence spectra of collagen sensitized by: (1) 10−5 M hypericin solution, (2) 2×10−5 M hypericin solution, and (3) 10−5 M hypericin solution after partial evaporation of water. λ exc =355 nm. ![]() Similar experiments were performed with 0.03 mL from chle 6 10 −5 M solution for comparison. It was visually obvious that the collagen samples were not stained by the chromophore, i.e., chle 6 could not penetrate into collagen fibrils and thus only interacted with the sample surface, in contrast to HYP. It was further shown that there was no spectral shifting or quenching of collagen fluorescence and there was no registration of chle 6 fluorescence from the collagen sample. However, characteristic chle 6 fluorescence was registered from the sample environment, when laser radiation was focused on the surrounding medium. Besides photophysical effects on collagen and gelatin, laser irradiation of samples revealed the existence of photochemical processes of potentially high importance, from a biological point of view. Laser irradiation at 355 nm of dry collagen and gelatin as well as of collagen in aqueous environment induced pronounced macromolecule photobleaching. This effect took place in a spectrally uniform manner, in the 385–450 nm spectral region. After interruption of irradiation neither recovery nor further decrease of the fluorescence intensity were observed. Thus the absence of any dark effect was obvious, while the decreasing of fluorescence continued after repeated irradiation. In Figure 6 the photobleaching is presented as a function of irradiation time. Figure 6Kinetics of dry collagen (1) and gelatin (2) fluorescence photobleaching under 355 nm laser irradiation. Average irradiation intensity −15 mW/cm2, F=10 Hz. λ fl =400 nm. ![]() The same experiment was performed with laser irradiation at 532 nm, to investigate the existence of photochemical processes induced to collagen by visible light, following the observed absorption and fluorescence in this spectral region. The result of laser irradiation of dry collagen, hydrated collagen, and HYP sensitized collagen at 532 nm with time can be seen in Figure 7. It is immediately obvious that there was photobleaching and that the effect was dependent on the surrounding medium. After irradiation of the HYP sensitized collagen sample in the UV or visible region, a profound decrease of intensity and alteration of the fluorescence spectral form took place as was shown earlier by the authors. 22 This alteration increased with irradiation time. The maximum of collagen fluorescence after photosensitization and 10 min of irradiation at 355 nm, shifted from 420 to 440 nm. In Figures 7 and 8 one can note the increase of photodestruction quantum efficiency of collagen fluorophores after HYP sensitization and irradiation at 532 nm. There was nonlinear correlation between irradiation intensity and photobleaching efficiency. With an intensity increase by a factor 2, 3, and 4, the initial rate of photobleaching increased by 1.65, 2, and 2.23 correspondingly (Figure 8). 4.DiscussionThere are numerous suggestions in the literature with regard to the nature of long wavelength collagen emission. Long wavelength photoluminescence of collagen and several other proteins with no tryptophan content was at first explained as phenylalanine phosphorescence. 1 However, it was demonstrated later 3 that collagen exhibited luminescence in the 400–700 nm spectral region with lifetime on the order of 10 ns. In the present work nanosecond laser excitation, in combination with the fast detection system, was used allowing the study of fluorescence but not phosphorescence. Hence, the photophysical processes observed occurred in singlet electron states of the molecules. Also, the absence of the characteristic fluorescence of tyrosine residues with maximum at 303 nm 1 is obvious in the case of collagen (Figure 2, curve 1). This characteristic fluorescence is however present in the emission spectrum of gelatin (curve 4), at 266 nm excitation. Similarly in the excitation spectra of dry gelatin one can see the contribution of tyrosine residues (Figure 4, curve 1), which is practically absent in the excitation spectrum of collagen at the same emission wavelength, namely 309 nm. It must be noted that the maximum of tyrosine absorption in aqueous solution lies at 275 nm, with a shoulder at 280 nm. 1 21 So, tyrosine fluorescence mechanism can be excluded. In Ref. 3 the authors suggested that collagen fluorescence was of excimer nature mainly attributed to phenylalanine residues as the fluorescence spectrum of collagen coincided with that of phenylalanine in powder form. In our work, nevertheless, long wavelength collagen excitation spectra, not characteristic of monomer phenylalanine, were registered. Hence it was safe to deduce that excimer formation cannot be the main mechanism for the explanation of the long wavelength (400–700 nm) collagen absorption and fluorescence. The dependence of emission spectra on excitation wavelengths and excitation spectra on emission wavelengths suggested the existence of several chromophores that absorb and fluoresce both in UV and visible spectral regions. In earlier work some age related collagen chromophores were isolated and identified as pentosidine and pyridinoline. 2 4 However, the absorption and fluorescence maxima of these chromophores lie below 400 nm. Our experimental results showed that pure collagen, both in dry and hydrated form, emits in the 600–700 nm spectral range at 532 nm excitation. Moreover, it was shown that certain chromophores can be very easily destroyed under green laser irradiation and they perhaps participate in important photochemical processes in collagen. The lifetime of the chromophore luminescence was less than 80 ns. The decrease of collagen photodestruction quantum efficiency with increasing irradiation pulse intensity, observed in Figure 8, can be attributed to the one-photon nature of the photodestruction mechanism and the saturation of the excited state of absorbing chromophores. Saturation was also responsible for the fact that, in our experiments, the photobleaching efficiency was 2–10 times less than that in related work, 8 9 10 11 where continuous-wave lasers or a nitrogen laser with no more than P=1 mJ/cm 2 pulse energy density were used. In the present work the corresponding value of pulse energy density was in the P=1.5–40 mJ/cm 2 range. Since this photobleaching was shown to be a one-photon irreversible effect, it can possibly be the result of long time exposure even to a low intensity light source. In our experiments, 9 J/cm2 at 355 nm and 18 J/cm2 at 532 nm irradiation were sufficient to induce a 30 photodegradation of pure collagen (Figures 6 and 7). The products of this photoreaction can interact with peptide bonds or aminoacid residues inducing important biochemical effects. From Figure 6 it is obvious that in aqueous medium the photobleaching efficiency slightly increased. It has been shown 11 that the photobleaching also was accentuated for collagen and reduced for elastin and cholesterol when the compounds were studied in hydrated form (λ exc =337 nm). However, the effect was small and could only be caused by physical factors, such as change in the polarity and the dielectric constant of the surrounding medium. 11 In addition to that, the considerable decrease of light scattering in aqueous environment also affected the observed photoprocesses. The irradiation of collagen and gelatin, under identical experimental conditions, showed that although the spatial structure of collagen quantitatively influenced the photobleaching efficiency, in general they photobleached in a similar manner. The obtained data confirmed observations of other authors that the tissue fluorescence photobleaching efficiency was higher in the case of UV irradiation, in comparison with excitation in the visible region. This was explained by less absorption and light scattering at longer wavelengths. 11 More essentially the energy of a UV photon being greater than that of a visible one, the probability of chemical bond breakage and chromophore destruction efficiency is also greater. Collagen fluorescence, spectrally nonuniform quenching by HYP (with shifting toward longer wavelengths) also verifies the existence of more than one chromophore in collagen. HYP can come into close range very easily and interact with collagen fluorophores responsible for its short wavelength fluorescence, while its quenching effect on fluorophores emission at longer wavelengths is not as profound. Chle 6 , on the other hand, does not seem to be able to come into close proximity with any of the collagen chromophores and thus does not practically affect collagen characteristic fluorescence or infer any photochemical changes. The sensitization of collagen with hypericin increased the efficiency of collagen photobleaching under both UV and visible irradiation. Although photobleaching of pure collagen was spectrally uniform under monochromatic laser irradiation without sensitization, in the case of HYP addition shifting of fluorescence toward longer wavelengths was observed. As it was shown in Ref. 22, the spectral shift of collagen fluorescence toward the longer wavelength region resembled the spectrum of denatured collagen (gelatin or heated collagen), indicating the breaking of crosslinks in collagen under the photointeraction with HYP. Further investigation is necessary to clarify whether there are conformational changes in collagen deriving from this interaction. Addition of hypocrellin to collagen solution produced results similar to ours, namely quenching of collagen fluorescence and shifting of its maximum. 23 The authors showed that the main mechanism responsible for fluorescence quenching was charge transfer. Since HYP has very similar structure and properties to hypocrellin, it is also our belief that processes of photoinduced charge transfer between HYP and collagen chromophores play an important role in photoinduced phenomena in HYP sensitized collagen. 5.ConclusionsIn the current work the existence of several types of chromophores absorbing and emitting throughout UV and visible spectral regions was observed. Laser irradiation at 355 and 532 nm was found to cause photobleaching of collagen fluorescence by 30, at the corresponding irradiation dozes of 9 and 18 J/cm2. These processes of collagen fluorophore photodestruction proved to be a one-photon effect. Addition of HYP aqueous solution to collagen produced quenching, redshift of the maximum, and broadening of the spectral form of its fluorescence. These effects became more prominent with increasing HYP concentration. The fluorescence of HYP sensitized collagen decreased in a spectrally nonproportional manner during laser irradiation at both 355 and 532 nm. The photobleaching effect was HYP concentration dependent. We believe that photobleaching is directly associated with the irreversible destruction of photolabile chromophores. In the case of visible light irradiation, certain collagen chromophore species that absorb in that spectral region were affected. The present studies of collagen fluorescence and photochemical behavior are of utmost biological importance. The observation of photobleaching under visible light irradiation may affect the contemporary philosophy of sun protection, which is focused in screening the UV electromagnetic radiation while maximal sun irradiation is around 500 nm. REFERENCES
A. Uchiyama
,
T. Ohishi
,
M. Takahashi
,
K. Kushida
,
T. Inoue
,
M. Fujieand
, and
K. Horiuchi
,
“Fluorophores from aging human cartilage,”
J. Biochem. , 110 714
–718
(1991). Google Scholar
A. S. Volkov
,
S. E. Kumekov
,
E. O. Syrgaliev
, and
S. V. Chernyshov
,
“Photoluminescence and anti-Stokes emission of native collagen in visible range of the spectrum,”
Biofizika , 36 770
–773
(1991). Google Scholar
D. Fujimoto
,
K. Akiba
, and
N. Nakamura
,
“Isolation and characterization of a fluorescent material in bovine Achilles tendon collagen,”
Biochem. Biophys. Res. Commun. , 76
(4), 1124
–1129
(1977). Google Scholar
J. M. Menter
,
J. D. Williamson
,
K. Carlyle
,
C. L. Moore
, and
I. Willis
,
“Photochemistry of type I acid soluble calf skin collagen: Dependence on excitation wavelength,”
Photochem. Photobiol. , 62
(3), 402
–408
(1995). Google Scholar
D. R. Cooper
and
R. J. Davidson
,
“The effect of ultraviolet irradiation on soluble collagen,”
Biochem. J. , 97 139
–147
(1965). Google Scholar
K. Sudoh
and
H. Noda
,
“UV irradiation of collagen and its effect on fibril reconstruction,”
Connect Tissue Res. , 1 267
–274
(1972). Google Scholar
H. W. Chaudhry
,
R. Richards-Kortum
,
T. Kolubayev
,
C. Kitrell
,
F. Partovi
,
J. R. Kramer
, and
M. S. Feld
,
“Alteration of spectral characteristics of human artery wall caused by 476 nm laser irradiation,”
Lasers Surg. Med. , 9 572
–580
(1989). Google Scholar
S. Andersson-Engels
,
J. Johansson
,
K. Svanberg
, and
S. Svanberg
,
“Fluorescence imaging and point measurements of tissue: applications to the demarcation of malignant tumors and atherosclerotic lesions from normal tissue,”
Photochem. Photobiol. , 53 807
–814
(1991). Google Scholar
D. J. Robinson
,
H. S. de Bruijn
,
N. van der Veen
,
M. R. Stringer
,
S. Brown
, and
W. M. Star
,
“Fluorescence photobleaching of ALA-induced protoporphyrin IX during photodynamic therapy of normal hairless mouse skin: The effect light dose and irradiance and the resulting biological effect,”
Photochem. Photobiol. , 67 140
–149
(1998). Google Scholar
L. Marcu
,
W. S. Grundfest
, and
J.-M. I. Maarek
,
“Photobleaching of arterial fluorescent compounds: characterization of elastin, collagen and cholesterol time-resolved spectra during prolonged ultraviolet irradiation,”
Photochem. Photobiol. , 69 713
–721
(1999). Google Scholar
S. Georgiou
,
T. Papazoglou
,
D. Dafnomili
,
A. G. Coutsolelos
,
V. Kouklaki
, and
A. J. Tosca
,
“Photophysical characterization of hematoporphyrin incorporated within collagen gels,”
J. Photochem. Photobiol., B , 22
(1), 45
–50
(1994). Google Scholar
S. M. Dets
,
A. Y. Joffe
,
A. N. Buryi
,
N. A. Denisov
,
I. S. Melnik
, and
A. H. Ravicz
,
“Hypericin-induced fluorescence in stomach cancer detection,”
173
–178
(1997). Google Scholar
S. Carpenter
and
G. A. Krauss
,
“Photosensitization is required for inactivation of equine infectious anemia virus by hypericin,”
Photochem. Photobiol. , 53 169
–174
(1991). Google Scholar
D. Meruelo
,
G. Lavie
, and
D. Lavie
,
“Therapeutic agents with dramatic antiretroviral activity and little toxicity at effective doses: Aromatic polycyclic diones hypericin and pseudohypericin,”
Proc. Natl. Acad. Sci. U.S.A. , 85 5230
–5234
(1998). Google Scholar
H. Koren
,
G. M. Schenk
,
R. H. Jindra
,
G. Alth
,
R. Ebermann
,
A. Kubin
,
G. Koderhold
, and
M. Kreitner
,
“Hypericin in phototherapy,”
J. Photochem. Photobiol., B , 36 113
–119
(1996). Google Scholar
J. M. Fernandez
,
M. D. Bilgin
, and
L. I. Grossweiner
,
“Singlet oxygen generation by photodynamic agents,”
J. Photochem. Photobiol., B , 37 131
–140
(1997). Google Scholar
D. S. English
,
K. Das
,
K. D. Ashby
,
J. Park
,
J. W. Petrich
, and
E. W. Castner
,
“Confirmation of excited-state proton transfer and ground-state heterogeneity in hypericin by fluorescence upconversion,”
J. Am. Chem. Soc. , 119 11585
–11590
(1997). Google Scholar
T. A. Wells
,
A. Losi
,
R. Dai
,
P. Scott
,
S. M. Park
,
J. Golbeck
, and
P. S. Song
,
“Electron transfer quenching and photoinduced EPR of hypericin and the ciliate photoreceptor stentorin,”
J. Phys. Chem. A , 101 366
–372
(1997). Google Scholar
A. Losi
,
“Fluorescence and time-resolved photoacoustics of hypericin inserted in liposomes: Dependence on pigment concentration and bilayer phase,”
Photochem. Photobiol. , 65 791
–801
(1997). Google Scholar
D. Yova
,
T. Theodossiou
, and
V. Hovhannisyan
,
“Photoinduced effect of hypericin on collagen and tissue with high collagen content,”
174
–180
(1999). Google Scholar
Y. H. Li
,
J. C. Yue
, and
G. P. Cai
,
“Fluorescence characterization of type I collagen from normal and silicotic rats and its quenching dynamics induced by hypocrellin B,”
Inc. Biopoly. , 42 219
–226
(1997). Google Scholar
|
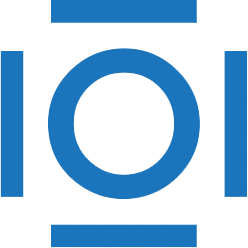
CITATIONS
Cited by 27 scholarly publications.
Collagen
Luminescence
Chromophores
Laser irradiation
Visible radiation
Ultraviolet radiation
Absorption