1.IntroductionFor the last few decades, optical spectro-imaging methods have been widely used in biology and medicine1. Among the wide range of applications, skin has become one of the main targeted structures, as their features such as non-invasiveness, high resolution, and speed of data acquisition made them a successful replacement or addition to traditional, often invasive and time-consuming methods, such as histological examinations. In particular, various spectroscopic methods, such as Raman, fluorescence, and diffuse reflectance spectroscopy, which allow quantitative and qualitative assessment of the biochemical structure of the studied tissues and their components, as well as imaging methods, such as confocal microscopy, multiphoton microscopy, second harmonic generation microscopy, and hyperspectral imaging, which allow for obtaining images of the studied tissues, are currently in clinical use for skin diagnostics and therapy.1–3 One of the widely used optical methods for skin imaging since the early 1990s is optical coherence tomography (OCT).4 This is an interferometric method in which the image of the tissue structure is obtained by registering low-coherent light backscattered or reflected by tissue.1,5 The original version of OCT was time-domain OCT (TD-OCT), which features fast translation of the internal interferometer’s reference mirror, allowing for the axial (i.e., perpendicular to skin surface) time-dependent scanning of skin.1 Full-field OCT was then developed, characterized by the ability to acquire “en face” (i.e., parallel to skin surface) skin images without the need to scan along the specimen.6 Another method is frequency- (or Fourier) domain OCT, featured by the absence of scanning of the reference mirror: scanning is carried out by the wavelengths of the source.7 Finally, a recently invented technique is line-field confocal OCT (LC-OCT) that combines the advantages of TD-OCT and confocal microscopy and provides 3D images of tissue at a sufficient resolution to distinguish the cellular structure of the skin.8 All techniques currently used in clinical environments for in vivo skin imaging at high spatial resolution do not properly meet the needs in dermatology for early non-invasive detection of all types of skin cancer (particularly melanoma) because of either insufficient resolution of the images (TD-OCT) or insufficient photon penetration depth in the tissue (in confocal and nonlinear microscopy). The lack of high-resolution vertically oriented cross-sectional views is also a limitation in confocal and nonlinear microscopy. Also, the use of OCT for skin imaging, as well as other optical techniques, is limited by strong light scattering by biological tissues.9 Thus, the resolution, contrast, and consequently, the diagnostic potential of this method are greatly reduced. The main reason for the strong scattering of biological tissues is the difference in the refractive indices (RI) of the tissue components and the interstitial fluid.1 Now, a widely investigated method to increase the refractive index of the interstitial fluid and thus reduce scattering is tissue optical clearing (OC).10,11 It is based on the use of chemical agents whose refractive indices are close to those of the structural components of biological tissue. Due to their immersion as a result of surface application or injection into biological tissue, they cause partial dehydration of the skin, and then replace the interstitial fluid. As a result of the action of these substances, called OC agents (OCAs), the scattering of biological tissues is reduced, which leads to an increase in the resolution and contrast of optical methods10,12,13. At the moment, there are many examples of using an OC technique to improve the contrast of OCT skin images in vivo10,11,14–16 and, in particular, its application for skin cancer characterization.17 However, when translating these methods into clinical use, there is a need to comply with established regulations, including the use of chemicals on healthy and pathologically changed skin of patients. At high concentrations, OCA show significant results; however, in clinical settings, the concentrations of the substances used may need to be reduced to pass the threshold of clinical utility and biocompatibility.18 The penetration of most chemicals is hindered, as they are stopped by the stratum corneum (SC) layer, which acts as a natural barrier of human body.19–21 Most of the used OCAs are hydrophilic and are used in high concentrations, which hinders their penetration through the SC into the living epidermis,22 but at the same time they may cause damage and even necrosis of the skin when injected under the dermis.23,24 However, a low concentration of OCA does not allow it to achieve a noticeable clearing effect. Thus, the use of OCA at biocompatible concentrations should be combined with the use of substances called chemical permeation enhancers (CPEs), which, even at relatively low allowed concentrations, can disrupt the impermeable structure of the SC and facilitate the penetration of OCA. In the literature, alcohols25 and organic solvents, such as dimethyl sulfoxide (DMSO),26,27 thiazone,28 and fatty acids (linoleic and oleic)26,29 are most often used as CPE. Additionally, a large number of physical methods for enhancing the skin permeability have been described in the literature.30–37 Among them, microdermabrasion31 and sonophoresis36,37 can be distinguished as minimally invasive. They can be combined with CPEs for more efficient biocompatible clearing. The aim of the current study was to experimentally evaluate the efficacy of optical clearing of human skin tissue in vivo using biocompatible OCA combined with chemical and physical permeability enhancers based on LC-OCT imaging. 2.Materials and Methods2.1.Chemical AgentsIn this study, nine different combinations of OCA and CPEs were studied as potential clinically biocompatible mixtures. The latter were chosen based on the literature data including our own preliminary studies.1,10,13,38 Three chemicals, most often referred to as OCA in the scientific literature, were selected: polyethylene glycol 400 (PEG, Sigma-Aldrich, United States) as an OCA from the group of alcohols and two aqueous 3M-solutions of sugars—sucrose (Sigma-Aldrich, United States) and glucose (Sigma-Aldrich, United States). Three other chemicals, mentioned as CPE in the scientific literature,1,13 were considered: propylene glycol (PG, Sigma-Aldrich, United States) as an alcohol group representative (DMSO, Sigma-Aldrich, United States) as an organic solvent and Oleic acid (OA, Sigma-Aldrich, United States) as the fatty acid. For the sake of biocompatibility, the concentrations of each of the aforementioned substances in the present experimental study did not exceed their maximum allowed concentration, established by the Food and Drug Administration (FDA) and contained in the inactive ingredients database, for topical application in the form of a solution.18 The resulting compositions of OCA and CPE, as well as their volume fractions and maximum allowed concentrations are shown in Table 1. As there is no information about maximum FDA-allowed concentration for topical application of glucose and sucrose, a concentration value (v/v) of 50% was established for our experiments as it was previously reported as the most effective concentration of glucose for OC.39 In the case of oleic acid and DMSO, it was not possible to mix each of them (alone) with any of the three OCA since in this case the maximum allowed concentration for these chemicals would be exceeded. For this purpose, PG or distilled water was additionally mixed as the second CPE. Table 1Concentration values of the nine mixtures of OCA and CPEs with corresponding FDA-allowed maximal concentrations for topical application in the form of solution and volume fractions (%, v/v) of resulting mixtures.
2.2.Anatomical Skin SitesThe human skin sites under investigation were the areas of left- and right-hand dorsum skin in vivo between the thumb and forefinger of healthy volunteers. Three volunteers aged between 25 and 31 years with the skin phototype of 2 and 3 were enrolled in this study. All skin areas were subjected to the experimental protocol repeatedly due to the number of mixtures and experimental conditions. To prevent residual effects of the previous protocol, each skin area remained intact for a week before the beginning of the next exposure. All volunteers gave their informed consent for topical application of OCAs mixtures, dermabrasion, and sonophoresis of skin dorsum of their hand and the acquisition of LC-OCT images, for the kinetics study. Volunteers’ safety was guaranteed thanks to FDA-approved concentrations of OCAs and to CE-marked LC-OCT, dermabrasion, and sonophoresis medical devices. An authorization for the human skin studies in vivo was obtained from the Saratov State Medical University Ethical Committee (protocol no. 11 by 7 June 2022). 2.3.Technical Equipment2.3.1.LC-OCTThe LC-OCT device “deepLive” (Damae Medical, France) was used for the image acquisition. Operating at a 650 to 950 nm spectral range emitted by a supercontinuum laser, it provides a unique 3D imaging modality, allowing one to switch from a histology-like vertical mode to a confocal-like horizontal mode and to record a 3D stack of tissue volumes in situ with a maximum axial and lateral resolution of and a penetration depth about .8 Such a resolution allows estimating the contrast changes of the tested skin caused by OC at a cellular level (Fig. 1). Fig. 1(a) Histology-like B-scan extracted from the LC-OCT acquired 3D image of human intact skin in vivo, displaying visible distinguishing between different skin layers. (b) Four different skin layers images, extracted as confocal-like horizontal scans from the 3D image: SC layer, acquired at depth (1), living epidermis, acquired at depth (2), papillary dermis, acquired at depth (3), and reticular dermis, acquired at depth (4). ![]() 2.3.2.Physical permeation enhancersTherapeutic ultrasound (US) (Pulson 100, Gymna, Belgium) was used as a physical permeability enhancer in experiments with OCT since this technique helps to increase the permeability of the skin.36,37,40 The duty cycle was 100%, frequency was 1 MHz, and the power density was . The second physical permeability enhancer was microdermabrasion (Philips VisaCare, Philips, Netherlands). This procedure is widely used in cosmetology and involves the abrasion/removing of the SC but does not damage the living epidermis that leads to increased penetration of OCA into the skin. It was recently shown in Ref. 37 that 1.5-min dermabrasion removed only of the SC; at the same time, erythema, as well as discomfort, were absent. 2.4.Experimental ProtocolThe skin sites were prior cleaned with ethanol to remove fat residuals. A starting LC-OCT 3D image of the latter cleaned intact skin was first taken ( time point) (Fig. 2). Then, the investigated area was treated for 1 min using a dermabrasion device followed by another 3D image acquisition. Afterwards, at time point , OCA was topically applied on the selected skin site, which was then exposed to therapeutic US for two consecutive sequences of 5 min duration each. Between and after the US treatments intervals (at and 11 min, respectively), and then every 5 min for 30 min (, 21, 26, 31, 36 and 41 min), 3D images of the selected area were taken using LC-OCT. Note that in those conditions the OCA mixture served as the immersion liquid for the LC-OCT probe during every successive image acquisition (including intact and after dermabrasion). Control measurements were performed at identical time points ( to 41 min) on a similar skin site only subjected to paraffin oil as the LC-OCT probe immersion liquid. The skin site in control measurements was not exposed to any of the external influences (neither dermabrasion nor sonophoresis) described in the protocol for OCA. 2.5.Data AnalysisFrom the obtained 3D images, the entire volume of in-depth data was taken for analysis, except for the superficial -thick layer. This was done to ignore the superficial areas of high contrast caused by reflection from the glass interface of the LC-OCT probe, which is configured with a slight slope. Thus, the -thick SC layer41 was mostly not included in the analysis, and the surface of the resulting image was represented by the stratum granulosum. For data analysis, the pixel intensity distribution was calculated for each horizontal section () of the 3D image (Fig. 3) along the -axis with a depth step of . Fig. 3Examples of individual horizontal sections extracted from LC-OCT 3D images at depth from intact skin (a) and from skin after OC (b); (c) corresponding graphs of the pixel intensity distribution in images at a depth for intact skin (black) and for skin after OC (red). Corresponding arrows show the FWHM for each curve. ![]() Note that the pixel intensity histograms obtained for most of the individual horizontal sections of 3D images had the form of a normal distribution, except for the uppermost skin layer images where secondary peaks appeared due to hollow black areas on images related to the skin relief (Fig. 4). The peak intensity and the full width at half maximum (FWHM) values of such a distribution make it possible to estimate, respectively, the average intensity and image contrast of an individual horizontal section. To simultaneously assess the effect of OC on the overall intensity and contrast of the horizontal section, the ratio between mean intensity and FWHM was calculated as Fig. 4Illustration of a typical 3D image of skin (a), acquired by LC-OCT (deepLive, Damae Medical, France) in the current study and corresponding “slices” of a surface (b) and in-depth sections (c) showing the presence of the skin relief-related hollow dark regions (green arrows). ![]() To estimate the ability and rate of OCA penetration into the superficial and deep layers of the skin, the relative changes in percentages of compared to the initial values (at ) were calculated for measurement steps , 11, and 26 min (Fig. 5), where is LC-OCT measurement after 5 min of US-assisted OC (OC+US), —after 10 minutes of OC+US, after OC. These ratios were calculated at 70, 250, and depths, in the midst of the living epidermis, the upper dermis, and the deep reticular dermis layers, respectively. Then, these ratios were averaged among the volunteers. Fig. 5Illustration of depth dynamic of ratio for different experimental protocol steps for one OCA, measured on one volunteer. The three vertical dotted black lines indicate the depths within the three different skin layers (shown with curly brackets) at which the changes of were calculated. ![]() Then, the area under curve (AUC) was calculated for the depth dependent graphs of in vivo skin ratio in the range 70 to . This value allows to evaluate the overall increase in contrast and intensity of the 3D image.1 The range between 0 and was excluded from AUC calculation because in the upper skin areas artifacts in the -values were observed, caused by the presence of the hollow areas in the horizontal sections described above. The obtained AUC values were averaged between the volunteers with respect to time points and OCA mixture. To compare the effectiveness of the different OCAs to each other as well as with control results, average AUC values previously calculated were all normalized to their corresponding initial values (at ), i.e., AUC relative values. Image processing and calculations were performed on MATLAB (R2018a, The Mathworks). 2.6.Exponential Fitting of AUC DataThe experimental protocol conducted in this study can be nominally divided in time into two phases. The first phase corresponds to the “active part” of the protocol in which all external manipulations (dermabrasion, US treatment) and, consequently, a major OC contribution were performed, and takes a time range from 0 to 11 min. The rest of the protocol involved only “passive” observation and measurements for 30 min (from to ), and thus the active manipulations here have already been completed and any changes in AUC can be mainly associated only with passive diffusion of OCA into the skin. To estimate the overall completion of the OC process after the end of the active phase, which would be an undoubted advantage of a specific OCA since in this case additional “passive” phase is not required, the experimental data were fitted. Taking into account the nominal separation of the experimental protocol into two phases described above, the biphasic exponential association was chosen as an appropriate model. It is described by the function where is the value at which exponential begins; and are the first and the second exponential amplitudes, respectively; and are the first and the second time offset, respectively; and and are the first exponential and the second exponential time constants, respectively.Since we assumed the hypothesis that the “passive” phase of our experimental protocol is a logical continuation of the “active,” and therefore, its amplitude should remain unchanged or increase (an insufficient time will pass for a significant reversal of the OC effect due to the in vivo physiological response), we determined for this model the lower bound of the parameter not lower than zero (). parameter was fixed as 1 for our model, as the fitted AUC data were normalized to initial value for all OCA. and parameters were manually fixed as 0 and 11 min, respectively, as the “active” phase starts at timepoint and the “passive” phase of our hypothesized biphasic model starts right after all the manipulations are done (). The fitting was performed in Origin 2018 (OriginLab Corporation). To compare simultaneously the amplitude and the time constant of the fitted kinetic AUC data, two ratios were calculated for each dataset. The first one is the slope at origin fitted curve for the first phase (), defined as . For the second “passive” exponential, an ratio was defined as . 3.Results and DiscussionThe average relative changes of values at three different skin depths for each experimental condition (each OCA) and for the control condition are presented in Fig. 6. The SD bars represent the standard deviation due to the biological variation of in vivo skin samples and also due to the fact that individual horizontal sections were used without averaging over the skin volume. Fig. 6Percentages of relative changes (with reference to initial value at ): after 5 min of OC and US (red) and after 10 min of OC and US (green) and 15 min after OC (blue). Average ± SD values are given among the volunteers for the 10 different OC mixtures: (a) glucose/DMSO, (b) glucose/OA/PG, (c) glucose/PG, (d) sucrose/DMSO, (e) sucrose/OA/PG, (f) sucrose/PG, (g) PEG/PG/DMSO, (h) PEG/OA/PG, (i) PEG/PG, and and (j) control condition. ![]() From the plots shown in Fig. 6, it can be observed that the control experiment [Fig. 6(j)], as expected, did not cause any significant increase in whatever the analyzed depth: mean change of at is . The use of DMSO [Figs. 6(a), 6(d) and 6(g)] as a CPE resulted in an increase in at depth (mean 27% increase at ). For mixtures of DMSO with both sugars, there was a rise in after the first 5 min of US-assisted OC (28% and 18%— increase for mixtures of glucose/DMSO and sucrose/DMSO, respectively), and then no significant increase in subsequent time periods (for glucose/DMSO, the ratio did not change 15 min after OC, for sucrose/DMSO we observed a 7% increase). Nevertheless, the use of PEG with DMSO in combination with PG as an additional CPE resulted in a 25% increase after 5 min of clearing, and at 15 min after clearing it was still possible to observe a 17% increase (up to a total 42% increase). A similar trend was observed in the deeper layers of the skin. At 250- and depths, the PEG/PG/DMSO mixture resulted in 24% and 20% increase, respectively, after 5 min of US clearing. Then, 15 min after clearing, the ratio rose again to 53% and 35%, respectively. The sucrose/DMSO mixture here also caused a clearing effect, expressed as an increase of the parameter, but on a smaller scale and without any increase with time. Remarkably, the glucose/DMSO mixture showed a very slight increase at a depth; however, at a depth, the ratio was modified (27% increase) after the second 5-min US clearing compared to the first one. This allows one to conclude that the dynamics of glucose/DMSO penetration into the deep layers of in vivo skin is lower compared to the PEG/PG/DMSO mixture. This is an interesting observation since the volume concentration of both sugars in OCA was several times higher than that of PEG. DMSO as CPE can interact with the lipid layers of the SC and thus facilitate the penetration of hydrophilic OCAs, such as sugars and PEG.1,13,42 It is likely that in our case the effect of DMSO on the increase in permeability in vivo was limited, which did not lead to a strong subsequent long-term increase in OC. The PG used in the PEG/PG/DMSO mixture is also able to interact with and dissolve SC lipids, accelerating skin dehydration under the action of hyperosmotic agents and increasing the rate of OC.10,43 Probably, the combination of these two CPEs made it possible to obtain an enhanced in-depth clearing effect that continued with time, even when using lower concentrations of the hyperosmotic agent. Moreover, PG and DMSO cannot be strictly classified as CPE since they also possess the properties of OCA.10,13 With the use of PG as CPE, the clearing effect for glucose and sucrose mixtures was mainly limited by the upper skin layers and did not increase much with time (at a depth, the change in , 15 min after OC, compared to the first 5 min of US clearing was 6% and 4% for mixtures of glucose/PG and sucrose/PG, respectively). Compared to sugar mixtures, the PEG/PG mixture showed a marked clearing effect even in the deep reticular dermis. At a depth, the ratio increased by 21% after 5 min of US-assisted clearing, and then, after 15 min, it continued to slightly increase to 28%. Compared to the PEG/PG/DMSO mixture results described above, the PEG/PG mixture showed clearing potential in the deep layers of in vivo skin, albeit on a smaller scale. This is probably due to the lack of penetration enhancing properties of DMSO,42 although PG was used in this case at a high (92% vol./vol,) concentration. Mixtures of sugars with a paired OA/PG used as CPE showed a better clearing effect in depth than with the other enhancers (mixed with DMSO). Even though the change in in the epidermal layer at a depth was not as significant as the one obtained with the other sugar/enhancer mixtures, at a depth, the mixtures of Glucose/OA/PG and Sucrose/OA/PG showed a steady increase during the experiment. For the Glucose/OA/PG mixture, this ratio continued to increase by 15% 15 min after the end of clearing compared to this value 5 min after US clearing. The similar increase for the sucrose/OA/PG mixture was observed with a value of 22%. Moreover, this trend was also found in the deep layers of the dermis. At depth, the relative change in the ratio for the Glucose/OA/PG mixture increased by a factor of almost 2.5 from 13% after 5 min of OC and US to 32% 15 min after the end of US-assisted clearing that is 25 min after OC start. The same values for a mixture of sucrose/OA/PG showed a three-fold increase from 7% to 21%. This difference from the results obtained without the use of oleic acid as CPE indicates the advantage of using it with the sugars to clear the “deep” layers of in vivo skin. OA, as well as DMSO and PG, can increase the permeability of the SC for OCA by disrupting the organization of its lipid layer.29,44,45 However, OA does not have hyperosmotic properties, and therefore does not cause much dehydration of the skin, unlike other CPEs. This fact, as well as, probably, a larger permeability increase of the SC layer leads to a deeper penetration of the OCA into the skin and, as a result, a better increase in the contrast and intensity of images throughout the depth. Based on the results presented in Fig. 6, the best OC effect across the depth of in vivo skin was achieved using a mixture of PEG/OA/PG. In the epidermal layer at a depth, the relative change between 15 min after OC and after 5 min of OC + US clearing was 26% (from 19% to 45%). This is the highest value of change achieved at this depth under the observed parameters. At the same time, in the dermis layer at depths of 250 and , the change in reached its maximum value already after 10-minutes of US-assisted clearing and amounted to 56% and 50%, respectively. Averaged ( volunteers) AUC kinetic curves of ratio (70 to ) and normalized to the corresponding initial values for each OC and control protocol are presented in Fig. 7. The SD bars were removed to keep the curves legible: the mean SD value is 10% of non-normalized initial values. Fig. 7Normalized mean AUC () variation kinetics of the ratio for the nine different OCA mixtures and for the control condition. ![]() These results confirm the observation and analysis conducted from Fig. 6. The PEG/OA/PG mixture showed the most confident kinetics and reached an AUC value of (corresponding to a 40% increase) already at the end of the 10-min clearing+US treatment. Then the changes were insignificant, which indicates the completion of the clearing process. The glucose/DMSO mixture showed a similar value at the same time point (), but as shown in Fig. 6, the clearing effect in the deeper skin layers was more pronounced when PEG/OA/PG was used as the OCA. These results are in good agreement with results referenced in Ref. 37, where the combined use of microdermabrasion, US-treatment, and oleic acid as an OCA on in vivo human skin resulted in more than a twofold increase in OCT probing depth and more than a threefold increase in OCT signal amplitude. Kinetics of AUC of ratio fitted with a biphasic exponential model showed high values of the coefficient of determination . Depending on the OCA used, took values in the range from 0.69 to 0.97 (Fig. 8). Fig. 8Experimental data (black circles) of the AUC of ratio time dependence and the corresponding fitted curves (red line) of the biphasic exponential model [Eq. (2)] for the mixture of (a) PEG/OA/PG, which showed the highest value (0.97) and for the mixture of (b) PEG/PG/DMSO, which showed the lowest value (0.69). In this model, the lower bound of the parameter was fixed to be not lower than zero (). parameter was fixed as 1, and and parameters were manually fixed as 0 and 11 min, respectively. ![]() The results of the biphasic exponential model-derived parameter analysis are presented in Fig. 9. Fig. 9Stacked histograms of (a) calculated and parameters and (b) derived and parameters of fitted curves of biphasic exponential association model. ![]() These results largely agree with the results presented in Figs. 6 and 7. The PEG/PG/DMSO mixture shows the highest stacked value of the SAO parameter; moreover, the main contribution to the column is made by the , which indicates the greatest efficiency of this OCA during the “active” phase of the biphasic exponential model in terms of the balance between achieved amplitude and the time spent on this. Quite similar results were achieved using the PEG/PG, sucrose/DMSO, and sucrose/PG mixtures. However, when looking at the stacked histograms of the exponential curves total amplitude, it becomes noticeable that for the above OCAs, a significant contribution to the amplitude is made by the parameter , which corresponds to the amplitude of the “passive” phase. Thus, their relatively small values and corresponding significant values indicate a long “passive” phase before the OC reaches its final plateau, which is not the optimal expected result. On the contrary, mixtures of glucose/DMSO, glucose/OA/PG, and PEG/OA/PG showed the highest values of the total amplitude, and the main contribution to the amplitude was made by parameter , which indicates their effectiveness in terms of the duration of the process—OC was for the most part completed within 11 min of the “active” phase, when all the manipulations described in the experimental protocol were completed. Comparison of the actual results of the AUC of ratio kinetics and the amplitudes of the exponential curve of the hypothesized biphasic model clearly shows that the PEG/OA/PG mixture has the best values of the increase in contrast and intensity of the signal obtained by LC-OCT in terms of the process time. Thus, we have experimentally shown the potential of OC of human skin in vivo, enhanced by chemical and physical methods of permeability enhancement, by biocompatible OCA, which makes it possible to safely use this technique for the diagnosis and treatment of healthy and pathologically altered skin areas. Further prospects for research in this direction should imply optimization of the biocompatible OCA and CPEs mixture composition by expanding the list of chemicals under study, as well as the inclusion of this technique in real clinical studies of neoplastic skin lesions. To improve the diagnostic ability of the optical method of skin analysis, it also makes sense to use a multimodal approach that combines OCT imaging technique and various methods of optical spectroscopy. This method also could be used for the improved lymphangiography of human in vivo skin in real time. 4.ConclusionWe presented the results of a comparative study of the OC effect by biocompatible mixtures of OCA and CPEs. Polyethylene glycol-400 as well as aqueous solutions of glucose and sucrose were used as OCA. Propylene glycol, oleic acid, and DMSO were used as CPEs. To enhance the clearing effect, physical permeation methods, such as dermabrasion and sonophoresis, were used. By analyzing the ratio, which contains information about the average intensity and contrast of the images obtained by LC-OCT method, we determined the in-depth effectiveness of skin in vivo OC using various OCA. The results showed that the overall level of the ratio in the 70- to skin depth region, calculated as the AUC, showed the best increase (40%) after 10 min of US-assisted clearing using a mixture of polyethylene glycol-400/oleic acid/propylene glycol. The other eight OCAs also showed an increase in the overall level with depth. The results of the experimental data fitting with the hypothesized biphasic exponential association model are in a good agreement with experimental results. Thus, the effectiveness of OC with biocompatible concentrations of OCA was proven through an increase in the overall intensity and contrast of the obtained LC-OCT images over the entire depth of the examined skin in vivo. AcknowledgementsSergey M. Zaytsev was supported by French Embassy in Russia under the Vernadski international joint PhD program of (2018 to 2021), and Russian Foundation for Basic Research (Grant No. 20-32-90043). Elina A. Genina was supported by the Russian Foundation for Basic Research (Grant No. 20-52-56005). Valery V. Tuchin was supported by grant under the Decree of the Government of the Russian Federation no. 220 of April 9, 2010 (Grant No. 075-15-2021-615 of June 4, 2021). Marine Amouroux and Walter Blondel acknowledge funding from CPER IT2MP (Contrat Plan Etat Région « Innovations Technologiques, Modélisation et Médecine Personnalisée »), the Ligue Contre le Cancer and the FEDER (Fonds Européen de Développement Régional) of the PhotoVivo platform and from the French Région Grand-Est, the Metz-Thionville Regional Hospital (2016 clinical research Award) in the frame of SpectroLive project. Data availabilityThe data that support the findings of this study are available from the authors on reasonable request. ReferencesV. V. Tuchin, Tissue Optics: Light Scattering Methods and Instruments for Medical Diagnosis, 3rd ed.SPIE, Bellingham, Washington
(2015). Google Scholar
V. Narayanamurthy et al.,
“Skin cancer detection using non-invasive techniques,”
RSC Adv., 8
(49), 28095
–28130 https://doi.org/10.1039/C8RA04164D
(2018).
Google Scholar
V. V. Tuchin, J. Popp and V. Zakharov, Multimodal Optical Diagnostics of Cancer, 1st ed.Springer, Cham
(2020). Google Scholar
D. Huang et al.,
“Optical coherence tomography,”
Science, 254
(5035), 1178
–1181 https://doi.org/10.1126/science.1957169 SCIEAS 0036-8075
(1991).
Google Scholar
J. G. Fujimoto, W. Drexler,
“Introduction to OCT,”
Optical Coherence Tomography: Technology and Applications, 3
–64 Springer, Cham
(2015). Google Scholar
F. Harms, A. Latrive, A. C. Boccara,
“Time domain full field optical coherence tomography microscopy,”
Optical Coherence Tomography: Technology and Applications, 791
–812 Springer, Cham
(2015). Google Scholar
J. F. de Boer,
“Spectral/Fourier domain optical coherence tomography,”
Optical Coherence Tomography: Technology and Applications, 165
–193 Springer, Cham
(2015). Google Scholar
J. Ogien et al.,
“Dual-mode line-field confocal optical coherence tomography for ultrahigh-resolution vertical and horizontal section imaging of human skin in vivo,”
Biomed. Opt. Express, 11
(3), 1327
–1335 https://doi.org/10.1364/BOE.385303 BOEICL 2156-7085
(2020).
Google Scholar
D. A. Boas,
“A fundamental limitation of linearized algorithms for diffuse optical tomography,”
Opt. Express, 1
(13), 404
–413 https://doi.org/10.1364/OE.1.000404 OPEXFF 1094-4087
(1997).
Google Scholar
V. V. Tuchin, D. Zhu and E. A. Genina, Handbook of Tissue Optical Clearing: New Prospects in Optical Imaging, 1st ed.CRC Press, Boca Raton
(2022). Google Scholar
L. M. C. Oliveira, V. V. Tuchin,
“Optical clearing and tissue imaging,”
The Optical Clearing Method: A New Tool for Clinical Practice and Biomedical Engineering, 107
–138 Springer, Cham
(2019). Google Scholar
A. Y. Sdobnov et al.,
“Recent progress in tissue optical clearing for spectroscopic application,”
Spectrochim. Acta A, 197 216
–229 https://doi.org/10.1016/j.saa.2018.01.085
(2018).
Google Scholar
E. A. Genina et al.,
“Tissue optical immersion clearing,”
Expert Rev. Med. Devices, 7
(6), 825
–842 https://doi.org/10.1586/erd.10.50 1743-4440
(2010).
Google Scholar
R. K. Wang and V. V. Tuchin,
“Enhance light penetration in tissue for high resolution optical imaging techniques by the use of biocompatible chemical agents,”
J. X-Ray Sci. Technol., 10
(3), 167
–176 JXSTE5 0895-3996
(2002).
Google Scholar
Y. M. Liew et al.,
“Reduction of image artifacts in three-dimensional optical coherence tomography of skin in vivo,”
J. Biomed. Opt., 16
(11), 116018 https://doi.org/10.1117/1.3652710 JBOPFO 1083-3668
(2011).
Google Scholar
R. He et al.,
“Effects of optical clearing agents on noninvasive blood glucose monitoring with optical coherence tomography: a pilot study,”
J. Biomed. Opt., 17
(10), 101513 https://doi.org/10.1117/1.JBO.17.10.101513 JBOPFO 1083-3668
(2012).
Google Scholar
L. Pires et al.,
“Optical clearing of melanoma in vivo: characterization by diffuse reflectance spectroscopy and optical coherence tomography,”
J. Biomed. Opt., 21
(8), 081210 https://doi.org/10.1117/1.JBO.21.8.081210 JBOPFO 1083-3668
(2016).
Google Scholar
“U. S. Food and Drug Administration, Inactive Ingredients Database,”
https://www.accessdata.fda.gov/scripts/cder/iig/index.cfm
().
Google Scholar
R. Yang et al.,
“Getting drugs across biological barriers,”
Adv. Mater., 29
(37), 1606596 https://doi.org/10.1002/adma.201606596 ADVMEW 0935-9648
(2017).
Google Scholar
C. Gorzelanny et al.,
“Skin barriers in dermal drug delivery: which barriers have to be overcome and how can we measure them?,”
Pharmaceutics, 12
(7), 684 https://doi.org/10.3390/pharmaceutics12070684
(2020).
Google Scholar
J. A. Bouwstra and M. Ponec,
“The skin barrier in healthy and diseased state,”
Biochim. Biophys. Acta, 1758
(12), 2080
–2095 https://doi.org/10.1016/j.bbamem.2006.06.021 BBACAQ 0006-3002
(2006).
Google Scholar
E. A. Genina et al.,
“In vivo skin optical clearing in humans,”
Handbook of Tissue Optical Clearing: New Prospects in Optical Imaging, 682 CRC Press, Boca Raton
(2022). Google Scholar
G. Vargas et al.,
“Morphological changes in blood vessels produced by hyperosmotic agents and measured by optical coherence tomography,”
Photochem. Photobiol., 77
(5), 541
–549 https://doi.org/10.1562/0031-8655(2003)077<0541:MCIBVP>2.0.CO;2 PHCBAP 0031-8655
(2003).
Google Scholar
V. V. Tuchin et al.,
“In vivo investigation of the immersion-liquid-induced human skin clearing dynamics,”
Tech. Phys. Lett., 27
(6), 489
–490 https://doi.org/10.1134/1.1383834 TPLEED 1063-7850
(2001).
Google Scholar
Z. Zhi et al.,
“Improve optical clearing of skin in vitro with propylene glycol as a penetration enhancer,”
J. Innov. Opt. Health Sci., 2
(3), 269
–278 https://doi.org/10.1142/S1793545809000590
(2009).
Google Scholar
Y. Liu et al.,
“Optical clearing agents improve photoacoustic imaging in the optical diffusive regime,”
Opt. Lett., 38
(20), 4236
–4239 https://doi.org/10.1364/OL.38.004236 OPLEDP 0146-9592
(2013).
Google Scholar
E. A. Genina et al.,
“Optical coherence tomography monitoring of enhanced skin optical clearing in rats in vivo,”
J. Biomed. Opt., 19
(2), 021109 https://doi.org/10.1117/1.JBO.19.2.021109 JBOPFO 1083-3668
(2013).
Google Scholar
J. Wang et al.,
“Switchable skin window induced by optical clearing method for dermal blood flow imaging,”
J. Biomed. Opt., 18
(6), 061209 https://doi.org/10.1117/1.JBO.18.6.061209 JBOPFO 1083-3668
(2012).
Google Scholar
J. Jiang and R. K. Wang,
“How different molarities of oleic acid as enhancer exert its effect on optical clearing of skin tissue in vitro,”
J. X-Ray Sci. Technol., 13
(3), 149
–159 JXSTE5 0895-3996
(2005).
Google Scholar
H. J. Weigmann et al.,
“Correlation of the local distribution of topically applied substances inside the stratum corneum determined by tape-stripping to differences in bioavailability,”
Skin Pharmacol. Appl., 14 98
–102 https://doi.org/10.1159/000056397
(2001).
Google Scholar
W. R. Lee et al.,
“Microdermabrasion as a novel tool to enhance drug delivery via the skin: an animal study,”
Dermatol. Surg., 32
(8), 1013
–1022 https://doi.org/10.1111/j.1524-4725.2006.32224.x
(2006).
Google Scholar
C. Tse et al.,
“Penetration and precision of subsurface photodisruption in porcine skin tissue with infrared femtosecond laser pulses,”
IEEE Trans. Bio-med. Eng., 55
(3), 1211
–1218 https://doi.org/10.1109/TBME.2008.915727
(2008).
Google Scholar
J. Yoon et al.,
“Enhancement of optical skin clearing efficacy using a microneedle roller,”
J. Biomed. Opt., 13
(2), 021103 https://doi.org/10.1117/1.2907483 JBOPFO 1083-3668
(2008).
Google Scholar
O. F. Stumpp et al.,
“Enhancement of transepidermal skin clearing agent delivery using a 980 nm diode laser,”
Laser. Surg. Med., 37
(4), 278
–285 https://doi.org/10.1002/lsm.20237
(2005).
Google Scholar
A. K. Nugroho et al.,
“Transdermal iontophoresis of rotigotine across human stratum corneum in vitro: influence of pH and NaCl concentration,”
Pharm. Res., 21
(5), 844
–850 https://doi.org/10.1023/B:PHAM.0000026438.57787.10 PHREEB 0724-8741
(2004).
Google Scholar
X. Xu and Q. Zhu,
“Sonophoretic delivery for contrast and depth improvement in skin optical coherence tomography,”
IEEE J. Sel. Top. Quantum. Electron., 14
(1), 56
–61 https://doi.org/10.1109/JSTQE.2007.912900 IJSQEN 1077-260X
(2008).
Google Scholar
E. A. Genina et al.,
“Rapid ultrasound optical clearing of human light and dark skin,”
IEEE Trans. Med. Imaging, 39
(10), 3198
–3206 https://doi.org/10.1109/TMI.2020.2989079 ITMID4 0278-0062
(2020).
Google Scholar
D. Zhu et al.,
“Recent progress in tissue optical clearing,”
Laser Photonics Rev., 7
(5), 732
–757 https://doi.org/10.1002/lpor.201200056
(2013).
Google Scholar
N. Sudheendran et al.,
“Assessment of tissue optical clearing as a function of glucose concentration using optical coherence tomography,”
J. Innov. Opt. Health Sci., 3
(3), 169
–176 https://doi.org/10.1142/S1793545810001039
(2010).
Google Scholar
B. E. Polat et al.,
“Ultrasound-mediated transdermal drug delivery: mechanisms, scope, and emerging trends,”
J. Control. Release, 152
(3), 330
–348 https://doi.org/10.1016/j.jconrel.2011.01.006 JCREEC 0168-3659
(2011).
Google Scholar
V. V. Tuchin,
“Tissue optics and photonics: light-tissue interaction,”
JBPE, 1
(2), 98
–134 https://doi.org/10.18287/JBPE-2015-1-2-98
(2015).
Google Scholar
A. N. C. Anigbogu et al.,
“Fourier transform Raman spectroscopy of interactions between the penetration enhancer dimethyl sulfoxide and human stratum corneum,”
Int. J. Pharm., 125
(2), 265
–282 https://doi.org/10.1016/0378-5173(95)00141-5 IJPHDE 0378-5173
(1995).
Google Scholar
V. Carrer et al.,
“Effect of propylene glycol on the skin penetration of drugs,”
Arch. Dermatol. Res., 312
(5), 337
–352 https://doi.org/10.1007/s00403-019-02017-5
(2020).
Google Scholar
J. Jiang and R. K. Wang,
“Comparing the synergistic effects of oleic acid and dimethyl sulfoxide as vehicles for optical clearing of skin tissue in vitro,”
Phys. Med. Biol., 49
(23), 5283
–5294 https://doi.org/10.1088/0031-9155/49/23/006 PHMBA7 0031-9155
(2004).
Google Scholar
S. M. Zaytsev et al.,
“Optimized skin optical clearing for optical coherence tomography monitoring of encapsulated drug delivery through the hair follicles,”
J. Biophotonics, 13
(4), e201960020 https://doi.org/10.1002/jbio.201960020
(2020).
Google Scholar
BiographySergey M. Zaytsev received his MS degree in physics from Saratov State University in 2018 and his PhD in signal and image processing from the University of Lorraine in 2022. Currently, he is a junior researcher at Saratov State University He is the author of 10 journal papers and 1 patent and has written a book chapter. His research interests include biological and medical physics, biomedical optics, optical measurements, and development of methods for control of tissue scattering properties. Marine Amouroux received her MS degree in bioengineering in 2005 and her PhD in life science in 2008 both from Université de Lorraine. Currently, she is a research engineer at CRAN laboratory, which is a joint research unit of Université de Lorraine and CNRS. She is the author of 2 patents, 2 book chapters, more than 15 communications in international conferences, and more than 10 journal papers. She has been a member of SPIE since 2015. Her research interests include translational research to bring biomedical innovations that she develops from laboratory to applied settings. Valery V. Tuchin is the director of the Science Medical Center and the head of the Department of Optics and Biophotonics at Saratov State University. He is a corresponding member of the RAS, a fellow of SPIE and OPTICA, and was awarded the Honored Science Worker of Russia, SPIE Educator Award, J.W. Goodman Book Writing Award, and Michael S. Feld Biophotonics Award. His research interests include tissue optics, laser medicine, tissue optical clearing, and nanobiophotonics. Elina A. Genina received her PhD and doctor of science degrees degrees in biophysics from Saratov State University in 2002 and 2017, respectively. Currently, she is a professor in the Departments of Optics and Biophotonics at Saratov State University. She is the coauthor of more than 150 journal papers, 13 patents, and 19 book chapters. She has 5363 citations and an h-index of 33 (Scopus, 2023). Her research interests include tissue optical properties, tissue optical clearing, photodynamic therapy, and plasmon resonance photothermal therapy. Walter Blondel received his MSc degree in optoelectronics and digital image processing from the University of Hertfordshire, England, in 1992 and his PhD in biomedical engineering from the Université Henri Poincaré, France, in 2000. Currently, he is a professor at the University of Lorraine, France. He is a member of SPIE. His research interests include UV-Vis-NIR optical spectroscopy and imaging instrumentation, clinical transfer, multidimensional spectroscopic data and image processing, and light–tissue interactions modeling. |
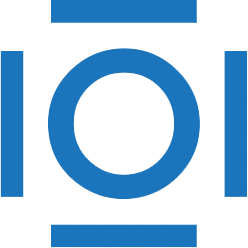
CITATIONS
Cited by 4 scholarly publications.
Skin
Mixtures
In vivo imaging
Optical clearing
Optical coherence tomography
Tissues
Confocal microscopy